The Mid-Pleistocene transition
The Mid-Pleistocene Transition (MPT) is also sometimes called "Early Middle Pleistocene Transition" or "Revolution" (Maasch, 1998; Berger and Jansen, 1994; Head and Gibbard, 2005). This period is characterized by a progressive increase of the climatic cycle amplitude, a shift from 41 ka to 100 ka of the climate response to orbital forcing and the apparition of a prominent asymmetry in large glacial-interglacial shifts, interglacial to glacial transitions being steep and glacial inceptions being more gradual. (Lisiecki and Raymo, 2005).
Many studies suggest that the transition was initiated at the Marine Isotopic Stage (MIS) 45, ~ 1.4 Ma ago, coinciding with a relative decrease in the duration of interglacials and an increase of glaciation amplitude. It was proposed that the end of the MPT corresponds to the MIS 12/11 transition (~ 0.4 Ma), which saw the onset of much higher amplitude interglacials in marine δ18O records than earlier in the MPT (Ruddiman et al., 1986; Lisiecki and Raymo, 2005; Lang and Wolff, 2011; Head and Gibbard, 2015). However, changes during the MPT could be more pronounced around the MIS 24-22 (900 ka; Head and Gibbard, 2015b and reference inside). For this reason, the exact timing and the duration of the MPT are still under debate, as are its abruptness and the interpretation of the changes in δ18O amplitude observed in marine carbonates between glacial and interglacial periods. Paleoclimatologists still discuss the role of orbital forcing and internal mechanisms involved.
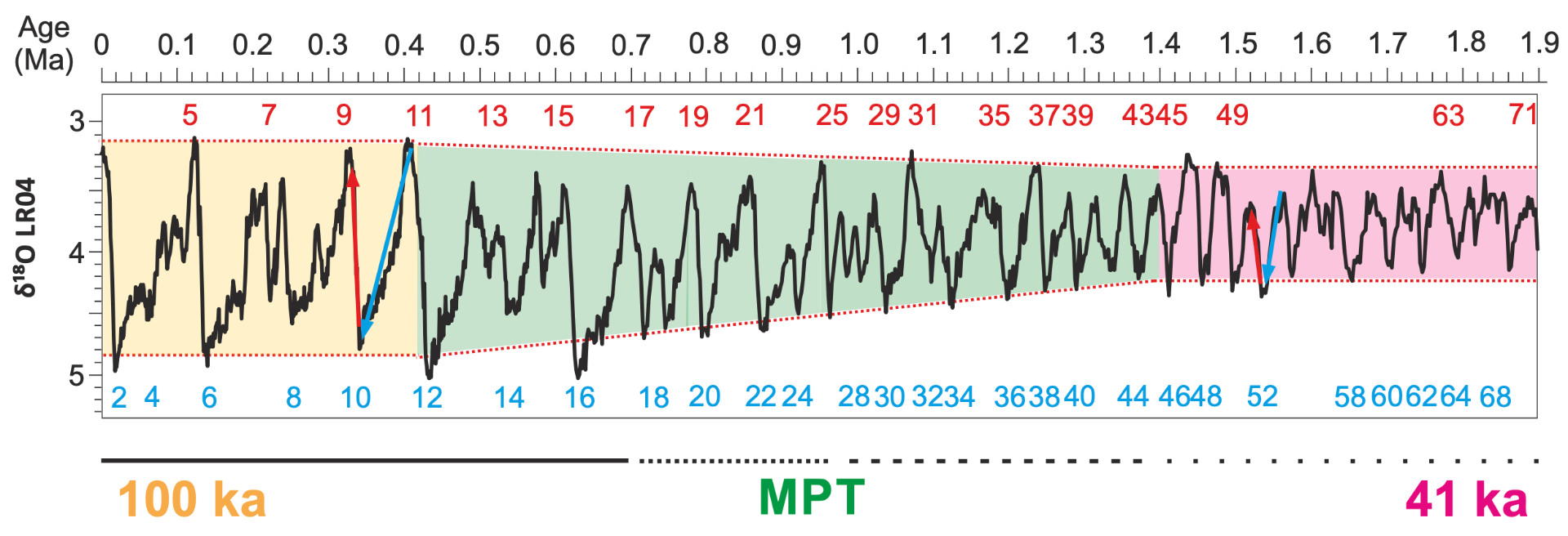
The MPT is marked by two major stratigraphic events:
- The interval from MIS 31 to MIS 28 is defined by the Jaramillo Subchron, a short-term geomagnetic reversal whose chronology is still under debate, but could correspond to the period from 1.070 to 0.988 Ma (i.e. Shackleton et al., 1990; Horng et al., 2002; Lourens et al., 2005; Hilgen et al., 2012).
- MIS 19 coincides with the Matuyama-Brunhes reversal (Head and Gibbard, 2015), while the age of the limit is still debated, around 770 - 780 ka (i.e. Shackleton et al., 1990; Bassinot et al., 1994; Lourens et al., 2005; Ferretti et al., 2015).
* From MIS 45 to MIS 25: stronger climatic contrast between glacial/interglacial periods
The beginning of the MPT is marked by an increase of dust and iron fluxes in the Southern Ocean (1.25 Ma - MIS 38) during glacials, resulting in strengthened ocean fertilisation and higher productivity (Martinez-Garcia et al., 2011). This fertilisation could have caused atmospheric CO2 concentration to decrease (Hönish et al., 2009; Chalk et al., 2017).
In parallel, marine data imply global sea surface cooling consistent with the development of ice sheets (Diekmann and Kuhn, 2002; Lisiecki and Raymo, 2005; Clark et al., 2006; McClymont et al., 2008, 2013; Sosdian and Rosenthal, 2009; Head and Gibbard, 2015). The expansion of polar water in glacial/interglacial periods could also be a consequence of this cooling. It has been suggested that intensification of the East Asia winter monsoon (Heslop et al., 2002; Ding et al., 2005; Sun et al., 2010; Elderfield et al., 2012; Malaizé et al., 2012), favouring arid conditions during glacial periods in North-West China, may have resulted an increase the latitudinal contrast between glacial and interglacial periods (Wright and Flower, 2002; Schefuß et al., 2003, 2005; McClymont et al., 2008; Hernandez-Almeida et al., 2012, 2013) due to high northern latitude cooling (Han et al., 2014; Kin et al., 2014). This strong latitudinal contrast could facilitate moisture transport to high latitudes and therefore the growth of ice sheets (Hernandez-Almeida et al., 2012).
One noticeable period in the early MPT is MIS 31 (1.081 to 1.062 Ma; Lisiecki and Raymo, 2005), called "super-interglacial" event (DeConto et al., 2012). It is characterized by strong insolation at high latitudes, and an unusual orbital configuration (high obliquity and eccentricity and minimal precession). This MIS records the highest summer insolation levels of the Pleistocene, with two southern hemisphere maxima bracketed by a northern hemisphere maximum at 1.070 Ma (Laskar et al., 2004). Models predict an eustatic rise of 20 m compared to present-day sea levels, probably due to retreats of Greenland and Antarctica ice sheets (Raymo et al., 2006). Atmospheric reconstructions for MIS 31 suggest high concentrations of greenhouse gas (Hönisch et al., 2009; Charlk and Hain et al., 2012). The δ18O, bottom-water temperatures and SST records are all consistent with warmer conditions than today (McClymont et al., 2008; Scherer et al., 2008; Elderfield et al., 2012; Melles et al., 2012) accompanied by a decrease of sea ice around Antarctica (Teitler et al., 2015) and possibly a short-term dissipation of the Polar front (Flores and Sierro, 2007; Maiorano et al., 2009; Villa et al., 2012).
* From MIS 24 to MIS 19: lengthening and intensification of glaciations
MIS 24, often called the 900 ka event (Clark et al., 2006) is the first long-lasting glaciation of the Pleistocene (McClymont et al., 2013). From MIS 24 to 22, several studies found evidence for anomalously cool SST worldwide (e.g. Schefuß et al., 2003, 2005; Marino et al., 2009, 2011; Kim et al., 2014; McClymont et al., 2013). This cooler period is accompanied by an intensification of Asian winter monsoons (Heslop et al., 2002; Dodonov, 2005), a reduction of deep-sea circulation (Schmieder et al., 2000; Ferretti et al., 2005; Venuti et al., 2007; Marino et al., 2009; Elderfield et al., 2012; Pena and Goldstein, 2014) and the development of polar ice sheets (Weirauch et al., 2008; Elderfield et al., 2012). From MIS 22 to 12, the Antarctic polar front and westerlies may shift northward, even during interglacials, resulting in a high ventilation of the deep ocean (Schefuß et al., 2003, 2005; deMenocal, 2004; Toggweiler et al., 2006; Kemp et al., 2010). This postulated shift may be related to substantial changes in the African monsoon system implying with alternation of arid and moist climates in North Africa, ad deposition of sapropels events in eastern Mediterranean during MIS 24 to 17 (Larrasoana, 2003; Kemp et al., 2010).
Intense Ice-Rafted Debris (IRD hereafter) events are observed in North Atlantic marine records for the glacial terminations MIS 24/23, 22/21 and 20/19 (Henandez-Almeida et al., 2012). Compared to earlier cold periods, MIS 22 and 20 are marked by an intensification of glacial conditions (Henandez-Almeida et al., 2012) and by large drops in sea level (- 120 m relative to present sea level; Elderfield et al., 2012). However, δ18O values in benthic foraminifera are higher for MIS 20 (0.814 Ma) than for MIS 22 (Lisiecki and Raymo, 2005; Elderfield et al., 2012; Henandez-Almeida et al., 2013). The increase of dust fluxes in the Eastern Mediterranean is evidence for weak African monsoons and extreme aridification in northeast Africa (Almogi-Labin, 2011). The MIS 20/19 termination is characterized by rapid eustatic rise (+ 20 m relative to present; Elderfield et al., 2012). MIS 19 is considered to be a good analogue of the current interglacial in terms of astronomical configuration and paleoclimatic records (Tzedakis et al., 2009, 2012; Tzedakis, 2010).
* From MIS 18 to MIS 11: high amplitude and asymmetrical variability
MIS 18 comprises two glacial phases separated by a weak interglacial (Railsback et al., 2015). This weak interglacial, MIS 17 and MIS 15 are all characterized by warm SST in southern oceans, and a southward displacement of the subtropical front (Marino et al., 2009).
MIS 16 is an unusually pronounced glacial period, marked by high concentration of IRD recorded in the North Atlantic (Lisiecki and Raymo, 2005; Hodell et al., 2008; Elderfield et al., 2012). The cooling phase supports the hypothesis that MIS 16 saw larger ice sheets than observed through the earlier MPT. These iceberg discharges could result from a weakening of thermohaline circulation in North Atlantic (Hodell et al., 2008).
MIS 13 is associated with exceptionally strong summer monsoon in the Northern Hemisphere (Schmieder et al., 2000). The high productivity observed during this stage in the Arabian Sea may be due to an intensification of the Atlantic meridional overturning circulation (Ziegler et al., 2010).
MIS 12 is one of the most extreme glacials across the MPT, with particularly high δ18O values and frequency climate variability (Lisiecki and Raymo, 2005; Weirauch et al., 2008; Elderfield et al., 2012). This stage is also marked by perturbations in deep-water circulation and Antarctic Bottom Water production (Venuti et al., 2007).
MIS 11 (0.424 to 0.374 Ma) is one of the longest and warmest interglacials of the past 400 ka (Droxler et al., 2003; Weirauch et al., 2008; Candy et al., 2014). It is comparable to the Holocene in terms of orbital configuration (Berger and Loutre, 2003; Tzedakis et al., 2012), sea level (Bowen, 2010) and global marine temperatures (Candy et al., 2014).
Different hypotheses for the origin of the MPT
Although orbital variations constitute the first-order forcing on glacial-interglacial oscillations of the late Quaternary, they cannot explain alone the shifts in climatic periodicity and amplitude observed during the MPT. Various hypotheses have been offered over the past decade regarding which internal mechanisms may explain this change in the global climatic response.
- Global temperatures cooling:
The MPT may have resulted from a global cooling trend (Zachos et al., 2001a). Numerous studies point to regional or global cooling over this period, which could allow larger ice sheets to persist in the late Pleistocene (Clark et al., 2006; Sosdian and Rossenthal, 2009, 2010; Herbert et al., 2010). Based on a world-wide SST stack, McClymont et al. (2013) documented a long-term cooling trend through the MPT, observable in both high and low latitudes, accompanied by a large increase in ice volume during glacials.
This global temperature change could be caused by (1) fertilization of oceans - the "iron hypothesis"; (2) the dissociation of gas hydrates; or (3) an acceleration of silicate weathering (3).
- Changes in heat distribution:
Tectonic processes may have an impact on the atmospheric and oceanic currents that could influence the global heat distribution. Atmospheric currents facilitate the transport of moisture from low to high latitudes and therefore the growth of ice sheets. In parallel, marine currents largely control the heat distribution in the world's ocean.
Continued progressive uplift of the Sierra Nevada, the Himalayas, and/or Tibetan plateau could have created a more Meridional atmospheric flow, favouring cooler summers in the high northern latitudes, reducing summer ablation of ice and inhibiting the northern ocean circulation (Ruddiman et al., 1986; Maslin et al., 2001).
- Progressive regolith erosion:
This hypothesis is based on the size and thickness increase of the
Laurentide ice sheet (Clark and Pollard, 1998; Clark et al., 2006; Sosdian and
Rosenthal, 2009). The increased resistance to melting in the context of an
"eccentricity world" could result from erosion, which caused the ice to rest
directly on the bedrock, leading to higher frictional resistance. Thus
stabilized, the Laurentide ice sheet could grow further before abruptly becoming
unstable after reaching a certain size, which would be consistent with the
first occurrence of IRD events in north Hemisphere Ocean and surrounding seas before
and after the MIS 25 (Hernandez-Almeida et al., 2012, 2013).